Introduction – Superconducting Qubits – Chemistry – Phase Noise
Majorana Fermions – Fluid Dynamics – In the Lab
This page is intended to be a non-technical introduction to quantum computing, and specifically my research projects. If you are familiar with the field, take a look at the papers listed on my CV.
A brief introduction to quantum computing

Despite the immense power of modern computers, there still remain problems they are fundamentally unable to solve; as the problem gets larger, the computational resources needed for a solution grows too fast–faster than you can throw more computers at it. (These problems are often called “classically intractable”.) One of these problems is at the heart quantum mechanics: the computational cost of solving a quantum system grows exponentially with the number of electrons in that system. In 1982, Richard Feynman famously suggested inverting the problem: since nature apparently performs these computations all the time, can we use quantum systems to solve difficult problems of our choosing? Since then–and especially since Peter Shor demonstrated in 1996 a quantum algorithm for factoring large numbers faster than the best known classical one–physicists the world over have been working to build a quantum computer.
The Martinis group and superconducting qubits

The Martinis group at UC Santa Barbara is developing a quantum computer made of superconducting qubits. Made of microscopic aluminum structures, at very low temperatures (less than one degree above absolute zero) they behave quantum mechanically, like well-controlled atoms. There are many approaches to building a quantum computer (actual atoms and ions, photons, and many more), each with their own advantages and disadvantages. Superconducting qubits are easy to manipulate and amenable to mass production (their fabrication is similar to how Intel makes a CPU), but they are susceptible to environmental noise; see the “Phase noise in superconducting qubits” section below.
Theoretical chemistry on a quantum computer
My thesis work has focused on demonstrating a practical, fully-scalable quantum chemistry algorithm–in particular, simulating molecules on a quantum computer in order to calculate energy surfaces. Quantum chemistry has been called the “killer app” for a quantum computer, as it seems likely that the first classically intractable problems to be solved on a QC will be chemistry simulations. Chemistry is, of course, immensely important industrially–after all, “Chemistry means the difference between poverty and starvation, and the abundant life.”

In particular, this work (published in the open-access journal Physical Review X) shows the first “fully-scalable” quantum algorithm for chemistry. This means that even though we only used a few qubits to simulate the simplest molecule of all (hydrogen), you could take the very same algorithm and run it on a quantum computer of dozens of qubits and make calculations about large molecules that you can’t do today on even the biggest supercomputers. Furthermore, we also demonstrated a relatively new hybrid quantum-classical algorithm (the variational quantum eigensolver, or VQE) that is tolerant of certain errors in the quantum computer.
The co-lead author on the paper, Ryan Babbush, posted an excellent entry on our work on the Google Research Blog, and it has also been picked up in a number of news outlets: Ars Technica, Chemistry World, Phys Org, The Register.
Phase noise in superconducting qubits

As mentioned above, superconducting qubits are susceptible to environmental noise, meaning that over the course of an algorithm the qubit loses its memory of its state. The working time of a qubit is known as its coherence time. While there are error correcting codes that could remove error from an algorithm at the cost of more qubits, and some algorithms (such as the VQE above) are robust to certain kinds of errors, all require a base level of performance, and higher quality qubits are always better.
Phase noise (or “dephasing” or “frequency noise”) is one of the major obstacles to building a quantum computer out of superconducting qubits. My first major superconducting qubit project was to characterize in detail this type of noise in our qubits. Whereas previously dephasing was measured over long timescales, we developed a method of characterizing it on the scale of quantum operations. The work is published in Physical Review Applied and is also available on the arXiv.
Majorana fermions
Prior to superconducting qubits, I worked on a project with the goal of realizing a new kind of qubit, the topological qubit. Such a qubit would be “topologically protected”–that is, immune to environmental noise, as long as you didn’t physically move it around (essentially impossible). These qubits would be constructed out of Majorana fermions, a theoretical particle proposed by the great Italian physicist Ettore Majorana, before he got on a boat to Naples and was never seen again.

The field of topological materials was (and still is) in its infancy, and this was a materials project, as the Majorana fermion requires getting a superconductor and a semiconductor to play nicely together in a magnetic field. The publication is here.
Inertial particles in fluid flows (my undergrad research)

As an undergraduate, I worked in the lab of Jerry Gollub at Haverford College. Jerry ran a fluid dynamics lab and is known for his work in chaos theory. My project was studying the behavior of large particles in a chaotic fluid flow. We found that while the statistics (velocity, distribution, etc.) of these particles were not significantly different from the flow they were in, their paths diverged dramatically from the fluid. The publication is here.
Hard at work…
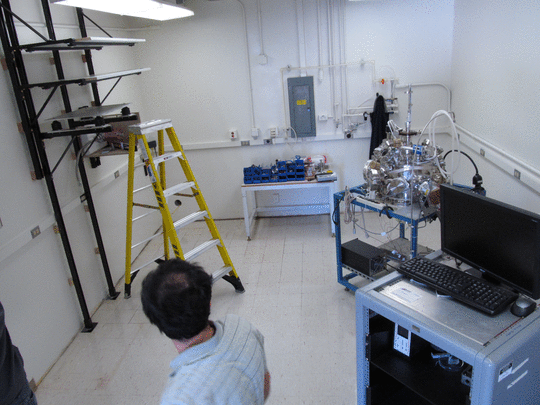